High throughput variant libraries and machine learning yield design rules for retron gene editors
- PMID:39658047
- PMCID: PMC11754653
- DOI: 10.1093/nar/gkae1199
High throughput variant libraries and machine learning yield design rules for retron gene editors
Abstract
The bacterial retron reverse transcriptase system has served as an intracellular factory for single-stranded DNA in many biotechnological applications. In these technologies, a natural retron non-coding RNA (ncRNA) is modified to encode a template for the production of custom DNA sequences by reverse transcription. The efficiency of reverse transcription is a major limiting step for retron technologies, but we lack systematic knowledge of how to improve or maintain reverse transcription efficiency while changing the retron sequence for custom DNA production. Here, we test thousands of different modifications to the Retron-Eco1 ncRNA and measure DNA production in pooled variant library experiments, identifying regions of the ncRNA that are tolerant and intolerant to modification. We apply this new information to a specific application: the use of the retron to produce a precise genome editing donor in combination with a CRISPR-Cas9 RNA-guided nuclease (an editron). We use high-throughput libraries in Saccharomyces cerevisiae to additionally define design rules for editrons. We extend our new knowledge of retron DNA production and editron design rules to human genome editing to achieve the highest efficiency Retron-Eco1 editrons to date.
© The Author(s) 2024. Published by Oxford University Press on behalf of Nucleic Acids Research.
Figures
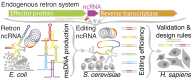
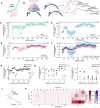
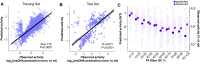
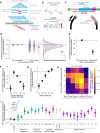
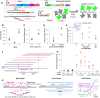
Update of
- High Throughput Variant Libraries and Machine Learning Yield Design Rules for Retron Gene Editors.Crawford KD, Khan AG, Lopez SC, Goodarzi H, Shipman SL.Crawford KD, et al.bioRxiv [Preprint]. 2024 Jul 9:2024.07.08.602561. doi: 10.1101/2024.07.08.602561.bioRxiv. 2024.Update in:Nucleic Acids Res. 2025 Jan 11;53(2):gkae1199. doi: 10.1093/nar/gkae1199.PMID:39026735Free PMC article.Updated.Preprint.
Similar articles
- High Throughput Variant Libraries and Machine Learning Yield Design Rules for Retron Gene Editors.Crawford KD, Khan AG, Lopez SC, Goodarzi H, Shipman SL.Crawford KD, et al.bioRxiv [Preprint]. 2024 Jul 9:2024.07.08.602561. doi: 10.1101/2024.07.08.602561.bioRxiv. 2024.Update in:Nucleic Acids Res. 2025 Jan 11;53(2):gkae1199. doi: 10.1093/nar/gkae1199.PMID:39026735Free PMC article.Updated.Preprint.
- Parallel CRISPR-Cas9 screens clarify impacts of p53 on screen performance.Bowden AR, Morales-Juarez DA, Sczaniecka-Clift M, Agudo MM, Lukashchuk N, Thomas JC, Jackson SP.Bowden AR, et al.Elife. 2020 May 22;9:e55325. doi: 10.7554/eLife.55325.Elife. 2020.PMID:32441252Free PMC article.
- Depressing time: Waiting, melancholia, and the psychoanalytic practice of care.Salisbury L, Baraitser L.Salisbury L, et al.In: Kirtsoglou E, Simpson B, editors. The Time of Anthropology: Studies of Contemporary Chronopolitics. Abingdon: Routledge; 2020. Chapter 5.In: Kirtsoglou E, Simpson B, editors. The Time of Anthropology: Studies of Contemporary Chronopolitics. Abingdon: Routledge; 2020. Chapter 5.PMID:36137063Free Books & Documents.Review.
- Improved gene editing and fluorescent-protein tagging inAspergillus nidulans using a Golden Gate-based CRISPR-Cas9 plasmid system.Modaffari D, Finlayson A, Miao Y, Wallace EWJ, Sawin KE.Modaffari D, et al.Wellcome Open Res. 2024 Oct 17;9:602. doi: 10.12688/wellcomeopenres.23086.1. eCollection 2024.Wellcome Open Res. 2024.PMID:39640368Free PMC article.
- The effectiveness of abstinence-based and harm reduction-based interventions in reducing problematic substance use in adults who are experiencing homelessness in high income countries: A systematic review and meta-analysis: A systematic review.O'Leary C, Ralphs R, Stevenson J, Smith A, Harrison J, Kiss Z, Armitage H.O'Leary C, et al.Campbell Syst Rev. 2024 Apr 21;20(2):e1396. doi: 10.1002/cl2.1396. eCollection 2024 Jun.Campbell Syst Rev. 2024.PMID:38645303Free PMC article.Review.
References
- Millman A., Bernheim A., Stokar-Avihail A., Fedorenko T., Voichek M., Leavitt A., Oppenheimer-Shaanan Y., Sorek R.. Bacterial retrons function in anti-phage defense. Cell. 2020; 183:1551–1561. - PubMed
MeSH terms
Substances
Related information
Grants and funding
LinkOut - more resources
Full Text Sources