Brain state identification and neuromodulation to promote recovery of consciousness
- PMID:39474045
- PMCID: PMC11520929
- DOI: 10.1093/braincomms/fcae362
Brain state identification and neuromodulation to promote recovery of consciousness
Abstract
Experimental and clinical studies of consciousness identify brain states (i.e. quasi-stable functional cerebral organization) in a non-systematic manner and largely independent of the research into brain state modulation. In this narrative review, we synthesize advances in the identification of brain states associated with consciousness in animal models and physiological (sleep), pharmacological (anaesthesia) and pathological (disorders of consciousness) states of altered consciousness in humans. We show that in reduced consciousness the frequencies in which the brain operates are slowed down and that the pattern of functional communication is sparser, less efficient, and less complex. The results also highlight damaged resting-state networks, in particular the default mode network, decreased connectivity in long-range connections and especially in the thalamocortical loops. Next, we show that therapeutic approaches to treat disorders of consciousness, through pharmacology (e.g. amantadine, zolpidem), and (non-) invasive brain stimulation (e.g. transcranial direct current stimulation, deep brain stimulation) have shown partial effectiveness in promoting consciousness recovery. Although some features of conscious brain states may improve in response to neuromodulation, targeting often remains non-specific and does not always lead to (behavioural) improvements. The fields of brain state identification and neuromodulation of brain states in relation to consciousness are showing fascinating developments that, when integrated, might propel the development of new and better-targeted techniques for disorders of consciousness. We here propose a therapeutic framework for the identification and modulation of brain states to facilitate the interaction between the two fields. We propose that brain states should be identified in a predictive setting, followed by theoretical and empirical testing (i.e. in animal models, under anaesthesia and in patients with a disorder of consciousness) of neuromodulation techniques to promote consciousness in line with such predictions. This framework further helps to identify where challenges and opportunities lay for the maturation of brain state research in the context of states of consciousness. It will become apparent that one angle of opportunity is provided through the addition of computational modelling. Finally, it aids in recognizing possibilities and obstacles for the clinical translation of these diagnostic techniques and neuromodulation treatment options across both the multimodal and multi-species approaches outlined throughout the review.
Keywords: (disorders of) consciousness; anaesthesia; animal models; brain states; neuromodulation.
© The Author(s) 2024. Published by Oxford University Press on behalf of the Guarantors of Brain.
Conflict of interest statement
The authors report no conflicts of interest.
Figures
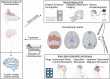
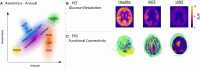
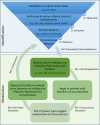
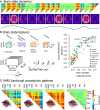
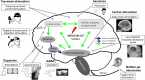
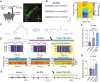
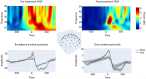
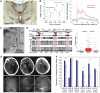
Similar articles
- Neural correlates of consciousness in patients who have emerged from a minimally conscious state: a cross-sectional multimodal imaging study.Di Perri C, Bahri MA, Amico E, Thibaut A, Heine L, Antonopoulos G, Charland-Verville V, Wannez S, Gomez F, Hustinx R, Tshibanda L, Demertzi A, Soddu A, Laureys S.Di Perri C, et al.Lancet Neurol. 2016 Jul;15(8):830-842. doi: 10.1016/S1474-4422(16)00111-3. Epub 2016 Apr 27.Lancet Neurol. 2016.PMID:27131917
- Current Status of Neuromodulatory Therapies for Disorders of Consciousness.Xia X, Yang Y, Guo Y, Bai Y, Dang Y, Xu R, He J.Xia X, et al.Neurosci Bull. 2018 Aug;34(4):615-625. doi: 10.1007/s12264-018-0244-4. Epub 2018 Jun 18.Neurosci Bull. 2018.PMID:29916112Free PMC article.Review.
- Neuroimaging and neuromodulation approaches to study eating behavior and prevent and treat eating disorders and obesity.Val-Laillet D, Aarts E, Weber B, Ferrari M, Quaresima V, Stoeckel LE, Alonso-Alonso M, Audette M, Malbert CH, Stice E.Val-Laillet D, et al.Neuroimage Clin. 2015 Mar 24;8:1-31. doi: 10.1016/j.nicl.2015.03.016. eCollection 2015.Neuroimage Clin. 2015.PMID:26110109Free PMC article.Review.
- Resting state networks and consciousness: alterations of multiple resting state network connectivity in physiological, pharmacological, and pathological consciousness States.Heine L, Soddu A, Gómez F, Vanhaudenhuyse A, Tshibanda L, Thonnard M, Charland-Verville V, Kirsch M, Laureys S, Demertzi A.Heine L, et al.Front Psychol. 2012 Aug 27;3:295. doi: 10.3389/fpsyg.2012.00295. eCollection 2012.Front Psychol. 2012.PMID:22969735Free PMC article.
- Neuromodulation and Disorders of Consciousness: Systematic Review and Pathophysiology.Dutta RR, Abdolmanafi S, Rabizadeh A, Baghbaninogourani R, Mansooridara S, Lopez A, Akbari Y, Paff M.Dutta RR, et al.Neuromodulation. 2025 Apr;28(3):380-400. doi: 10.1016/j.neurom.2024.09.003. Epub 2024 Oct 18.Neuromodulation. 2025.PMID:39425733Review.
References
- Nagel T. What is it like to be a bat ? Philos Rev. 1974;83:435–450.
Publication types
Related information
LinkOut - more resources
Full Text Sources