Practical Guide to Measuring Wetland Carbon Pools and Fluxes
- PMID:38037553
- PMCID: PMC10684704
- DOI: 10.1007/s13157-023-01722-2
Practical Guide to Measuring Wetland Carbon Pools and Fluxes
Abstract
Wetlands cover a small portion of the world, but have disproportionate influence on global carbon (C) sequestration, carbon dioxide and methane emissions, and aquatic C fluxes. However, the underlying biogeochemical processes that affect wetland C pools and fluxes are complex and dynamic, making measurements of wetland C challenging. Over decades of research, many observational, experimental, and analytical approaches have been developed to understand and quantify pools and fluxes of wetland C. Sampling approaches range in their representation of wetland C from short to long timeframes and local to landscape spatial scales. This review summarizes common and cutting-edge methodological approaches for quantifying wetland C pools and fluxes. We firstdefine each of the major C pools and fluxes and providerationale for their importance to wetland C dynamics. For each approach, we clarifywhat component of wetland C is measured and its spatial and temporal representativeness and constraints. We describe practical considerations for each approach, such aswhere andwhen an approach is typically used,who can conduct the measurements (expertise, training requirements), andhow approaches are conducted, including considerations on equipment complexity and costs. Finally, we reviewkey covariates andancillary measurements that enhance the interpretation of findings and facilitate model development. The protocols that we describe to measure soil, water, vegetation, and gases are also relevant for related disciplines such as ecology. Improved quality and consistency of data collection and reporting across studies will help reduce global uncertainties and develop management strategies to use wetlands as nature-based climate solutions.
Supplementary information: The online version contains supplementary material available at 10.1007/s13157-023-01722-2.
Keywords: Accretion; Accumulation; Biomass; Bulk density; Carbon cycling; Chambers; Core; Decomposition; Dissolved gas; Dissolved organic carbon; Eddy covariance; Greenhouse gas; Groundwater; Hydrology; Incubation; Lateral transport; Litter; Methane; Methods; Microbes; Models; Net primary productivity; Plants; Porewater; Radiometric dating; Remote sensing; Sediment; Soil organic carbon; Vegetation; Water.
© The Author(s) 2023.
Conflict of interest statement
Competing InterestsThe authors declare that the research was conducted in the absence of any commercial or financial relationships that could be construed as a potential conflict of interest.
Figures
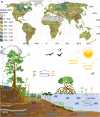
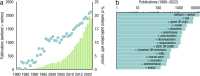
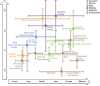
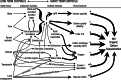
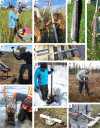
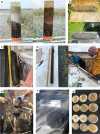
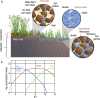
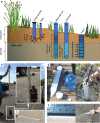
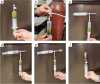
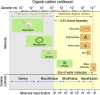
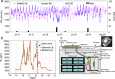
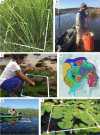
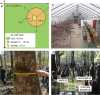
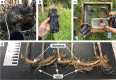
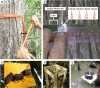
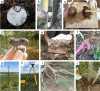
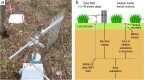
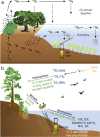
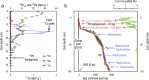
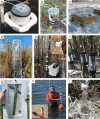
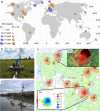
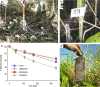
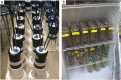
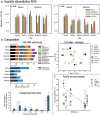
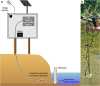
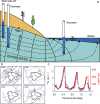
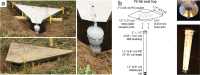
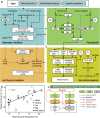
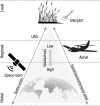
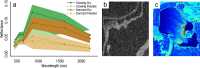
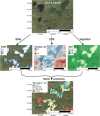
Similar articles
- Water level changes in Lake Erie drive 21st century CO2 and CH4 fluxes from a coastal temperate wetland.Morin TH, Riley WJ, Grant RF, Mekonnen Z, Stefanik KC, Sanchez ACR, Mulhare MA, Villa J, Wrighton K, Bohrer G.Morin TH, et al.Sci Total Environ. 2022 May 15;821:153087. doi: 10.1016/j.scitotenv.2022.153087. Epub 2022 Jan 15.Sci Total Environ. 2022.PMID:35038507
- Soil properties and sediment accretion modulate methane fluxes from restored wetlands.Chamberlain SD, Anthony TL, Silver WL, Eichelmann E, Hemes KS, Oikawa PY, Sturtevant C, Szutu DJ, Verfaillie JG, Baldocchi DD.Chamberlain SD, et al.Glob Chang Biol. 2018 Sep;24(9):4107-4121. doi: 10.1111/gcb.14124. Epub 2018 Apr 10.Glob Chang Biol. 2018.PMID:29575340
- Effects of seasonality, transport pathway, and spatial structure on greenhouse gas fluxes in a restored wetland.McNicol G, Sturtevant CS, Knox SH, Dronova I, Baldocchi DD, Silver WL.McNicol G, et al.Glob Chang Biol. 2017 Jul;23(7):2768-2782. doi: 10.1111/gcb.13580. Epub 2017 Jan 7.Glob Chang Biol. 2017.PMID:27888548
- Global nitrogen input on wetland ecosystem: The driving mechanism of soil labile carbon and nitrogen on greenhouse gas emissions.Chen M, Chang L, Zhang J, Guo F, Vymazal J, He Q, Chen Y.Chen M, et al.Environ Sci Ecotechnol. 2020 Oct 13;4:100063. doi: 10.1016/j.ese.2020.100063. eCollection 2020 Oct.Environ Sci Ecotechnol. 2020.PMID:36157707Free PMC article.Review.
- Wetland phosphorus dynamics and phosphorus removal potential.Skinner M.Skinner M.Water Environ Res. 2022 Oct;94(10):e10799. doi: 10.1002/wer.10799.Water Environ Res. 2022.PMID:36259138Review.
Cited by
- A Low-Cost Sensor Network for Monitoring Peatland.Mitchell HL, Cox SJ, Lewis HG.Mitchell HL, et al.Sensors (Basel). 2024 Sep 18;24(18):6019. doi: 10.3390/s24186019.Sensors (Basel). 2024.PMID:39338763Free PMC article.
- New perspectives on temperate inland wetlands as natural climate solutions under different CO2-equivalent metrics.Ma S, Creed IF, Badiou P.Ma S, et al.NPJ Clim Atmos Sci. 2024;7(1):222. doi: 10.1038/s41612-024-00778-z. Epub 2024 Sep 28.NPJ Clim Atmos Sci. 2024.PMID:39430200Free PMC article.
References
- Abella SR, Zimmer BW. Estimating organic carbon from loss-on-ignition in northern Arizona forest soils. Soil Science Society of America Journal. 2007;71:545–550. doi: 10.2136/sssaj2006.0136. - DOI
- Åberg J, Wallin B. Evaluating a fast headspace method for measuring DIC and subsequent calculation of pCO2 in freshwater systems. Inland Waters. 2014;4:157–166. doi: 10.5268/IW-4.2.694. - DOI
- Abril G, Bouillon S, Darchambeau F, et al. Technical Note: Large overestimation of pCO2 calculated from pH and alkalinity in acidic, organic-rich freshwaters. Biogeosciences. 2015;12:67–78. doi: 10.5194/bg-12-67-2015. - DOI
- Abril JM. A new theoretical treatment of compaction and the advective-diffusive processes in sediments: A reviewed basis for radiometric dating models. Journal of Paleolimnology. 2003;30:363–370. doi: 10.1023/B:JOPL.0000007220.16908.d4. - DOI