Swarming magnetic nanorobots bio-interfaced by heparinoid-polymer brushes for in vivo safe synergistic thrombolysis
- PMID:38019908
- PMCID: PMC10686566
- DOI: 10.1126/sciadv.adk7251
Swarming magnetic nanorobots bio-interfaced by heparinoid-polymer brushes for in vivo safe synergistic thrombolysis
Abstract
Biocompatible swarming magnetic nanorobots that work in blood vessels for safe and efficient targeted thrombolytic therapy in vivo are demonstrated. This is achieved by using magnetic beads elaborately grafted with heparinoid-polymer brushes (HPBs) upon the application of an alternating magnetic fieldB(t). Because of the dense surface charges bestowed by HPBs, the swarming nanorobots demonstrate reversible agglomeration-free reconfigurations, low hemolysis, anti-bioadhesion, and self-anticoagulation in high-ionic-strength blood environments. They are confirmed in vitro and in vivo to perform synergistic thrombolysis efficiently by "motile-targeting" drug delivery and mechanical destruction. Moreover, upon the completion of thrombolysis and removal ofB(t), the nanorobots disassemble into dispersed particles in blood, allowing them to safely participate in circulation and be phagocytized by immune cells without apparent organ damage or inflammatory lesion. This work provides a rational multifaceted HPB biointerfacing design strategy for biomedical nanorobots and a general motile platform to deliver drugs for targeted therapies.
Figures
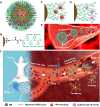
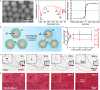
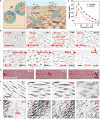
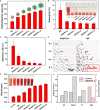
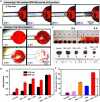
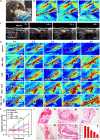
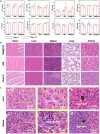
Similar articles
- Dual Frequency-Regulated Magnetic Vortex Nanorobots Empower Nattokinase for Focalized Microvascular Thrombolysis.Gao R, Zhang W, Chen X, Shen J, Qin Y, Wang Y, Wei X, Zou W, Jiang X, Wang Y, Huang W, Chen H, Li Z, Fan H, He B, Cheng Y.Gao R, et al.ACS Nano. 2024 Oct 29;18(43):29492-29506. doi: 10.1021/acsnano.4c04331. Epub 2024 Oct 18.ACS Nano. 2024.PMID:39422644
- Erratum: Preparation of Poly(pentafluorophenyl acrylate) Functionalized SiO2 Beads for Protein Purification.[No authors listed][No authors listed]J Vis Exp. 2019 Apr 30;(146). doi: 10.3791/6328.J Vis Exp. 2019.PMID:31038480
- Swarming Multifunctional Heater-Thermometer Nanorobots for Precise Feedback Hyperthermia Delivery.Liu J, Li L, Cao C, Feng Z, Liu Y, Ma H, Luo W, Guan J, Mou F.Liu J, et al.ACS Nano. 2023 Sep 12;17(17):16731-16742. doi: 10.1021/acsnano.3c03131. Epub 2023 Aug 31.ACS Nano. 2023.PMID:37651715
- Micro/nanorobots for precise drug delivery via targeted transport and triggered release: A review.Xu Y, Bian Q, Wang R, Gao J.Xu Y, et al.Int J Pharm. 2022 Mar 25;616:121551. doi: 10.1016/j.ijpharm.2022.121551. Epub 2022 Feb 5.Int J Pharm. 2022.PMID:35131352Review.
- Engineering Nanorobots for Tumor-Targeting Drug Delivery: From Dynamic Control to Stimuli-Responsive Strategy.Huang L, Chen F, Lai Y, Xu Z, Yu H.Huang L, et al.Chembiochem. 2021 Dec 10;22(24):3369-3380. doi: 10.1002/cbic.202100347. Epub 2021 Sep 14.Chembiochem. 2021.PMID:34411411Review.
Cited by
- Magnetic Microrobot Swarms with Polymeric Hands Catching Bacteria and Microplastics in Water.Ussia M, Urso M, Oral CM, Peng X, Pumera M.Ussia M, et al.ACS Nano. 2024 May 21;18(20):13171-13183. doi: 10.1021/acsnano.4c02115. Epub 2024 May 8.ACS Nano. 2024.PMID:38717036Free PMC article.
- The Model Study of Phase-Transitional Magnetic-Driven Micromotors for Sealing Gastric Perforation via Mg-Based Micropower Traction.Xiong K, Xu L.Xiong K, et al.Nanomaterials (Basel). 2024 May 16;14(10):865. doi: 10.3390/nano14100865.Nanomaterials (Basel). 2024.PMID:38786822Free PMC article.
- Harnessing Disparities in Magnetic Microswarms: From Construction to Collaborative Tasks.Cao C, Mou F, Yang M, Zhang S, Zhang D, Li L, Lan T, Xiao D, Luo W, Ma H, Guan J.Cao C, et al.Adv Sci (Weinh). 2024 Aug;11(30):e2401711. doi: 10.1002/advs.202401711. Epub 2024 Jun 13.Adv Sci (Weinh). 2024.PMID:38868929Free PMC article.Review.
- Bio-Hybrid Magnetic Robots: From Bioengineering to Targeted Therapy.Zhang Q, Zeng Y, Zhao Y, Peng X, Ren E, Liu G.Zhang Q, et al.Bioengineering (Basel). 2024 Mar 26;11(4):311. doi: 10.3390/bioengineering11040311.Bioengineering (Basel). 2024.PMID:38671732Free PMC article.Review.
- Swarming Magnetic Fe3O4@Polydopamine-Tannic Acid Nanorobots: Integrating Antibiotic-Free Superficial Photothermal and Deep Chemical Strategies for Targeted Bacterial Elimination.Si L, Zhang S, Guo H, Luo W, Feng Y, Du X, Mou F, Ma H, Guan J.Si L, et al.Research (Wash D C). 2024 Jul 31;7:0438. doi: 10.34133/research.0438. eCollection 2024.Research (Wash D C). 2024.PMID:39086398Free PMC article.
References
- D. Jin, K. Yuan, X. Du, Q. Wang, S. Wang, L. Zhang, Domino reaction encoded heterogeneous colloidal microswarm with on-demand morphological adaptability. Adv. Mater. 33, e2100070 (2021). - PubMed
- Q. Wang, L. Zhang, External power-driven microrobotic swarm: From fundamental understanding to imaging-guided delivery. ACS Nano 15, 149–174 (2021). - PubMed
MeSH terms
Substances
Related information
LinkOut - more resources
Full Text Sources