Structural basis for ion permeation mechanism in pentameric ligand-gated ion channels
- PMID:23403925
- PMCID: PMC3590989
- DOI: 10.1038/emboj.2013.17
Structural basis for ion permeation mechanism in pentameric ligand-gated ion channels
Abstract
To understand the molecular mechanism of ion permeation in pentameric ligand-gated ion channels (pLGIC), we solved the structure of an open form of GLIC, a prokaryotic pLGIC, at 2.4 Å. Anomalous diffraction data were used to place bound anions and cations. This reveals ordered water molecules at the level of two rings of hydroxylated residues (named Ser6' and Thr2') that contribute to the ion selectivity filter. Two water pentagons are observed, a self-stabilized ice-like water pentagon and a second wider water pentagon, with one sodium ion between them. Single-channel electrophysiology shows that the side-chain hydroxyl of Ser6' is crucial for ion translocation. Simulations and electrostatics calculations complemented the description of hydration in the pore and suggest that the water pentagons observed in the crystal are important for the ion to cross hydrophobic constriction barriers. Simulations that pull a cation through the pore reveal that residue Ser6' actively contributes to ion translocation by reorienting its side chain when the ion is going through the pore. Generalization of these findings to the pLGIC family is proposed.
Conflict of interest statement
The authors declare that they have no conflict of interest.
Figures
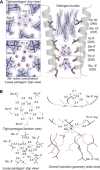
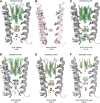
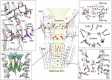
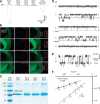
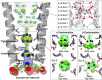
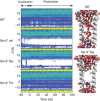
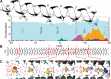
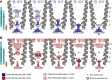
Similar articles
- Functional characterization of two prokaryotic pentameric ligand-gated ion channel chimeras - role of the GLIC transmembrane domain in proton sensing.Hénault CM, Baenziger JE.Hénault CM, et al.Biochim Biophys Acta Biomembr. 2017 Feb;1859(2):218-227. doi: 10.1016/j.bbamem.2016.11.006. Epub 2016 Nov 12.Biochim Biophys Acta Biomembr. 2017.PMID:27845033
- Structural basis for allosteric transitions of a multidomain pentameric ligand-gated ion channel.Hu H, Howard RJ, Bastolla U, Lindahl E, Delarue M.Hu H, et al.Proc Natl Acad Sci U S A. 2020 Jun 16;117(24):13437-13446. doi: 10.1073/pnas.1922701117. Epub 2020 Jun 1.Proc Natl Acad Sci U S A. 2020.PMID:32482881Free PMC article.
- Ion selectivity mechanism in a bacterial pentameric ligand-gated ion channel.Fritsch S, Ivanov I, Wang H, Cheng X.Fritsch S, et al.Biophys J. 2011 Jan 19;100(2):390-8. doi: 10.1016/j.bpj.2010.11.077.Biophys J. 2011.PMID:21244835Free PMC article.
- A gating mechanism of pentameric ligand-gated ion channels.Calimet N, Simoes M, Changeux JP, Karplus M, Taly A, Cecchini M.Calimet N, et al.Proc Natl Acad Sci U S A. 2013 Oct 15;110(42):E3987-96. doi: 10.1073/pnas.1313785110. Epub 2013 Sep 16.Proc Natl Acad Sci U S A. 2013.PMID:24043807Free PMC article.Review.
- An outline of desensitization in pentameric ligand-gated ion channel receptors.Keramidas A, Lynch JW.Keramidas A, et al.Cell Mol Life Sci. 2013 Apr;70(7):1241-53. doi: 10.1007/s00018-012-1133-z. Epub 2012 Aug 31.Cell Mol Life Sci. 2013.PMID:22936353Free PMC article.Review.
Cited by
- Prediction and validation of protein intermediate states from structurally rich ensembles and coarse-grained simulations.Orellana L, Yoluk O, Carrillo O, Orozco M, Lindahl E.Orellana L, et al.Nat Commun. 2016 Aug 31;7:12575. doi: 10.1038/ncomms12575.Nat Commun. 2016.PMID:27578633Free PMC article.
- Allosteric binding site in a Cys-loop receptor ligand-binding domain unveiled in the crystal structure of ELIC in complex with chlorpromazine.Nys M, Wijckmans E, Farinha A, Yoluk Ö, Andersson M, Brams M, Spurny R, Peigneur S, Tytgat J, Lindahl E, Ulens C.Nys M, et al.Proc Natl Acad Sci U S A. 2016 Oct 25;113(43):E6696-E6703. doi: 10.1073/pnas.1603101113. Epub 2016 Oct 10.Proc Natl Acad Sci U S A. 2016.PMID:27791038Free PMC article.
- Structural analysis of pathogenic missense mutations in GABRA2 and identification of a novel de novo variant in the desensitization gate.Sanchis-Juan A, Hasenahuer MA, Baker JA, McTague A, Barwick K, Kurian MA, Duarte ST; NIHR BioResource; Carss KJ, Thornton J, Raymond FL.Sanchis-Juan A, et al.Mol Genet Genomic Med. 2020 Jul;8(7):e1106. doi: 10.1002/mgg3.1106. Epub 2020 Apr 29.Mol Genet Genomic Med. 2020.PMID:32347641Free PMC article.
- X-ray structures of GluCl in apo states reveal a gating mechanism of Cys-loop receptors.Althoff T, Hibbs RE, Banerjee S, Gouaux E.Althoff T, et al.Nature. 2014 Aug 21;512(7514):333-7. doi: 10.1038/nature13669.Nature. 2014.PMID:25143115Free PMC article.
- Subcellular neuronal quasicrystals: Implications for consciousness.Gardiner J.Gardiner J.Commun Integr Biol. 2015 May 6;8(2):e983760. doi: 10.4161/cib.32161. eCollection 2015 Mar-Apr.Commun Integr Biol. 2015.PMID:26478770Free PMC article.
References
- Abascal JL, Sanz E, Garcia Fernandez R, Vega C (2005) A potential model for the study of ices and amorphous water: TIP4P/Ice. J Chem Phys 122: 234511. - PubMed
- Amiri S, Tai K, Beckstein O, Biggin PC, Sansom MS (2005) The alpha7 nicotinic acetylcholine receptor: molecular modelling, electrostatics, and energetics. Mol Membr Biol 22: 151–162 - PubMed
- Beckstein O, Sansom MS (2006) A hydrophobic gate in an ion channel: the closed state of the nicotinic acetylcholine receptor. Phys Biol 3: 147–159 - PubMed
- Blanc E, Roversi P, Vonrhein C, Flensburg C, Lea SM, Bricogne G (2004) Refinement of severely incomplete structures with maximum likelihood in BUSTER-TNT. Acta Crystallogr D Biol Crystallogr 60: 2210–2221 - PubMed
Publication types
MeSH terms
Substances
Related information
LinkOut - more resources
Full Text Sources
Other Literature Sources
Molecular Biology Databases