the Creative Commons Attribution 4.0 License.
the Creative Commons Attribution 4.0 License.
Increasing coastal slump activity impacts the release of sediment and organic carbon into the Arctic Ocean
Retrogressive thaw slumps (RTSs) are among the most activethermokarst landforms in the Arctic and deliver a large amount of materialto the Arctic Ocean. However, their contribution to the organic carbon (OC)budget is unknown. We provide the first estimate of the contribution of RTSsto the nearshore OC budget of the Yukon Coast, Canada, and describe theevolution of coastal RTSs between 1952 and 2011 in this area. We (1) describethe evolution of RTSs between 1952 and 2011; (2) calculate the volume oferoded material and stocks of OC mobilized through slumping, including soilorganic carbon (SOC) and dissolved organic carbon (DOC); and (3) estimate the OCfluxes mobilized through slumping between 1972 and 2011. We identified RTSsusing high-resolution satellite imagery from 2011 and geocoded aerialphotographs from 1952 and 1972. To estimate the volume of eroded material,we applied spline interpolation on an airborne lidar dataset acquired inJuly 2013. We inferred the stocks of mobilized SOC and DOC from existingrelated literature. Our results show a 73 % increase in the number of RTSsand 14 % areal expansion between 1952 and 2011. In the study area, RTSsdisplaced at least16.6×106 m3 of material, 53 % of which wasice, and mobilized145.9×106 kg of OC. Between 1972 and 2011, 49 RTSsdisplaced8.6×103 m3 yr−1 of material, adding 0.6 % to the OC fluxreleased by coastal retreat along the Yukon Coast. Our results show that thecontribution of RTSs to the nearshore OC budget is non-negligible and shouldbe included when estimating the quantity of OC released from the Arcticcoast to the ocean.
- Article
(6889 KB) - Full-text XML
- Supplement
(221 KB) - BibTeX
- EndNote
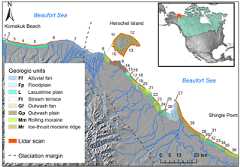
Figure 1Study area. The coastal subset defined as the lidar scan isrepresented in red. The limit of the glaciation was reproduced after Dyke andPrest (1987) and the surficial sediments after Rampton (1982). The numbersstand for the coastal segments stretching along the coast from west to east(the names of the coastal segments are available in the Supplement, Table S1).
Soil organic carbon (SOC) stocks in the top 3 m of soils, in deltas and theYedoma regions across the northern circumpolar permafrost region areestimated to 1307 Pg; 76.4 % (999 Pg) of them are stored in perenniallyfrozen soils (Hugelius et al., 2014). These stocks resulted from the slowdecomposition of soil organic matter in permanently frozen soils, caused bylow soil temperatures and impeded drainage. Surface air temperature in theArctic increased by 0.755 ∘C per decade during 1998–2012 (Huang etal., 2017). As the active layer, the upper part of the permafrost that thawsduring summer and refreezes in winter, thickens due to warmer air, increasedmicrobial activity in the soil mobilizes more organic carbon (OC) that iseventually released to the atmosphere (Mackelprang et al., 2011; Schuur etal., 2008). Organic carbon and nutrients are also released to streams,rivers, and to the Arctic Ocean by thermokarst and thermo-erosional processes(Schuur et al., 2015; Abbott and Jones, 2015; Kokelj et al., 2013; Vonk etal., 2012; Ping et al., 2011; Lamoureux and Lafrenière, 2009). Permafrostcarbon stocks were only recently included in calibrating global carbonmodels, highlighting a relevant contribution of thawing permafrost to theoverall climate and economic response to human greenhouse gas emissions(Kessler, 2017; Koven et al., 2015; MacDougall et al., 2012; Burke et al.,2012; Schneider von Deimling et al., 2012). Schaefer et al. (2014) predicted120±85 Gt carbon emissions from thawing permafrost by 2100, whichrepresents5.7±4.0 % of the total anthropogenic emissions.Nevertheless, these carbon models underestimate the potential impact of thepermafrost feedback on the global climate because they do not account for thespatial heterogeneity of permafrost terrains and omit the contribution ofcoastal erosion and abrupt thaw processes, such as thermokarst andthermo-erosion (Hugelius et al., 2014; MacDougall et al., 2012; Vonk et al.,2012). Both expert assessments (Abbott et al., 2016) and model evaluations(McGuire et al., 2016) identified permafrost degradation as one of the mostimportant sources of uncertainty in predicting the timing and magnitude ofthe permafrost carbon feedback.
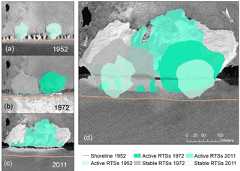
Figure 2Geomorphological map of retrogressive thaw slumps (RTSs)illustrating the complexity of RTS evolution along the Yukon Coast.(d) RTSsidentified in 1952 (a) and in 1972 (b) overlap the 2011 RTSs (c).The underlying imagery is a GeoEye-1 satellite image from 2011 (18 July).RTS areas from 1952 and 1972 closer to the shore have eroded due tocoastal retreat. The remaining parts have either extended and merged withother RTSs or stabilized in 2011.(a) Two active RTSs in 1952 (aerial photofrom 1952, National Air Photo Library, Canada).(b) RTSs in 1972 (aerialphoto from 1972, National Air Photo Library, Canada). RTSs expanded and onehad stabilized. New active RTSs developed within the stabilized RTS.(c) RTSsin 2011 (GeoEye-1, 18 July 2011). Former RTSs had partly stabilizedand newer RTSs developed within the boundaries of the stabilized RTSs.
Thermokarst and thermo-erosional processes occur by the thawing of ice-richpermafrost and the melting of massive ice. Thermokarst landscapes cover up to20 % of the northern circumpolar permafrost region and store half of theSOC from this region (Olefeldt et al., 2016). Retrogressive thaw slumps (RTSs), a type of slope failurecaused by permafrost thaw, are among the most active landforms in the Arcticand have increased both in number and size over the past decades (Ramage etal., 2017; Segal et al., 2016; Brooker et al., 2014; Lacelle et al., 2010).RTSs rework sediments and mobilize carbon, nitrogen, and nutrients; as aresult, RTSs affect terrestrial (Cassidy et al., 2016; Tanski et al., 2016;Cray and Pollard, 2015; Cannone et al., 2010) and aquatic ecosystems (Maloneet al., 2013; Kokelj et al., 2009a, 2013). Along the coasts of the Arctic,RTSs directly contribute to the transport of terrestrial OC to the nearshorezone (Obu et al., 2016), which has the potential to affect the nearshoremarine ecosystem (Fritz et al., 2017). However, there are currently noestimates on the volume of sediments and thus on the OC displaced by RTSsfrom the land to the nearshore zone in the Arctic. To provide betterestimates of the contribution of abrupt thaw processes on the OC budget alongArctic coasts, our study quantifies the impact of RTSs onthe OC budget in a coastal permafrost environment along the Yukon Coast,Canada. We (1) describe the evolution of RTSs in the area between 1952 and2011; (2) calculate the volume of material eroded and stocks of organiccarbon (OC) mobilized through slumping – including soil organic carbon (SOC)and dissolved organic carbon (DOC) – and (3) estimate the OC fluxesmobilized through slumping between 1972 and 2011.
The study area is located in the Canadian Arctic, along the westernmostcoast of the Yukon Territory (Fig. 1). The study area comprises a 238 kmportion of the Yukon Coastal Plain, including Herschel Island (Fig. 1). Thearea is in the continuous permafrost zone (Rampton, 1982) and tundravegetation zone dominated by mosses, graminoids, and shrubs (CAVM Team,2003). The area is characterized by a subarctic climate with a mean summer airtemperature of 6 ∘C on the eastern boundary of the study area and 8.7 ∘C on the western boundary; the mean summer precipitation(June, July, and August, 1971–2000) is 79.8 mm at the east end and 112.9 mmat the west end (Environment Canada, 2017). The Mackenzie River, whichenters the Beaufort Sea east of the study area, influences seawatertemperature and sea ice extent and is the main control on the localprecipitation patterns (Burn and Zhang, 2009). The western margin of theLaurentide ice sheet, which reached its maximum ice extent around HerschelIsland at ca. 16 200 years BP (Fritz et al., 2012), shaped the topography ofthe Yukon Coastal Plain. Long and high moraine ridges characterize most ofthe previously glaciated area. Herschel Island is a moraine thrust at themargin of the formerly glaciated area and is one of the largest morainedeposits in the region (Mackay, 1959). Stream valleys, fluvial deltas,alluvial fans, and thermokarst basins characterize the unglaciated area. Dueto widespread moraine deposits, 35 % of the Yukon Coast is composed ofice-rich cliffs (Harper, 1990). Volumetric ground ice contents (massive ice,pore ice, and wedge ice) vary along the coast and range from 0 to 74 %(Couture and Pollard, 2017). Previous studies divided the study area into 36coastal segments (Fig. 1), based on ground ice contents, surficial geology, and geomorphology (Lantuit et al., 2012b; Couture, 2010; Lantuit andPollard, 2005). Most segments fall into one of three surficial geologicunits: ice-thrust moraines (30 %), lacustrine plains (23 %), and rollingmoraines (16 %). Alluvial fans, stream terraces, floodplains, and outwashplains underlay the remaining segments (Rampton, 1982). The coast is rapidlyeroding (Harper, 1990): during the period 1952–2011, the average rate ofshoreline change was−0.7 m yr−1 and was characterized by decreasing erosionrates from west to east (Irrgang et al., 2018). RTSs are common along thecoast and mostly develop on segments with massive ground ice thicker than1.5 m and coastal slope greater than 3.9∘ (Ramage et al., 2017).
3.1Evolution of RTSs
We used two data inputs to measure the evolution of RTSs between 1952 and2011: a dataset with RTSs present in 1972 and 2011 (dataset A) and a datasetwith RTSs present in 1952 (dataset B). All RTSs were mapped using ArcMap10.3 (ESRI) on a scale of1:2000 and classified as active or stable. ActiveRTSs are characterized by steep headwalls exposing ice-rich permafrost,slump floors with thawed sediments, and incised gullies. Stable RTSscomprise gently sloping and vegetated headwalls, vegetated slump floors, andno visible active gully systems (Ramage et al., 2017; Lantuit and Pollard,2008; Wolfe et al., 2001).
Ramage et al. (2016) provided dataset A. RTSs present in 2011 were mappedbased on multispectral GeoEye-1 and WorldView-2 satellite images acquired inJuly, August, and September 2011. RTSs present in 1972 were mapped using aseries of geocoded aerial photographs from the 1970s obtained from theNational Air Photo Library in Canada (Irrgang et al., 2018). The mappingmethodology is explained in detail in Ramage et al. (2017).
Dataset B comprises RTSs present in 1952 that we mapped using a series ofgeocoded aerial photographs from the 1950s, obtained from the National AirPhoto Library in Canada (Irrgang et al., 2018).
We compared the number and size of RTSs present in 1952, in 1972, and in2011. RTSs are polycyclic and can occur on surfaces previously affected byRTSs. As a result, several active RTSs can be located within the boundary ofa stable RTS (Fig. 2). In this case, stable polycyclic RTSs include theareal surfaces of active RTSs located within their boundaries.
3.2Volume estimations
3.2.1Lidar dataset
For each RTS identified in 2011 we extracted morphological information –size and mean surface elevation – from an airborne lidar dataset acquiredin July 2013 (Kohnert et al., 2014). The lidar dataset has a scan width of500 m; the lidar point data were interpolated with inverse distance weightingto obtain digital elevation models with a horizontal resolution of 1 m (Obuet al., 2016). The lidar dataset has a final georeferenced point cloud datavertical accuracy of0.15±0.1 m and covers 80 % of the coastlinein our study area.
We selected a subset of the 2011 RTS dataset comprising RTSs that occurredwithin the boundary of the lidar dataset to measure the volume of erodedmaterial from RTSs (Fig. 1). We discarded the 125 RTSs outside of the lidarscan from the volume and flux analyses.
In addition to the RTSs present in 2011 within the lidar area, we defined asubgroup with RTSs present in 2011 on surfaces not affected by slumpingbefore 1972; we defined this subgroup asRTSs initiated after 1972.
3.2.2Interpolation method
We applied a regularized spline interpolation technique to model pre-slumptopographies used for calculating the volume of material eroded throughslumping. The spline method allows us to estimate elevation points outside therange of input sample points and to minimize the total curvature of thesurface. We therefore selected spline among other interpolation methods. Webased our interpolation on the extensive point elevation data available forthe study area from the lidar dataset (Fig. 3).
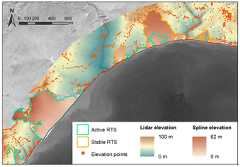
Figure 3Map illustrating the different datasets used to model pre-slumptopographies. Retrogressive thaw slumps (RTSs) are outlined in green for theactive RTSs and orange for the stable RTSs. The background satellite imageryis a GeoEye-1 image taken on 18 July 2011. The background elevationand the random elevation points outside the RTS areas are derived from thelidar dataset. Elevation surface within the RTS borders represents theelevation before RTS occurred and is interpolated using a splineinterpolation.
3.2.3Volume of eroded material
To calculate the volume of eroded material from the retrograding headwall ofthe RTSs identified in 2011, we subtracted the mean surface elevation valuesobtained from the lidar dataset from the mean interpolated surface elevationvalues (Fig. 3). However, these volumes do not account for the materialeroded from the RTS headwalls that settles within the RTS floors and for thematerial eroded and transported alongshore by coastal processes (Fig. 4).Due to ground ice melting, ca. 5.5 % of the reworked sediments subside andremain compacted in the RTS floor, i.e. do not get transported out of theRTS (Obu et al., 2016). We therefore adjusted the material volumes to takeinto account the 5.5 % of the material that subside in the RTS floors(Fig. 4c). In addition, we calculated the volumes of material eroded andtransported by coastal erosion using the rate of shoreline change between1952 and 2011 from Irrgang et al. (2018). Using this rate, we calculated thevolumes of eroded material between 1952 and 2011 for each RTS. For the RTSsthat initiated after 1972, we calculated the volumes of eroded materialbetween 1972 and 2011 (Fig. 4d).
To differentiate between the volumes of ice and sediments eroded, we usedthe volumetric ice content provided for each coastal segment in Couture andPollard (2017). The model interpolates the data collected on 19 coastalsegments to the whole Yukon Coast based on similarities between surficialgeology and permafrost conditions. Ice contents were determined from shallowcores collected from upper soil layers and from bluff exposures.
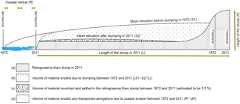
Figure 4Cross section of a retrogressive thaw slump (RTS)(a) illustrating thecalculated volumes of sediments eroded through slumping between 1972 and2011. The calculation estimates the amount of material released to thenearshore zone through slumping(b) and takes into account the materialeroded from the RTS headwalls that remains within the RTS floors where itsettles(c) and(d) the material eroded and transported alongshore bycoastal erosion. The volume of material that remains within the RTS floorswas estimated from Obu et al. (2016).
3.3Estimates of soil and dissolved organic carbon values
We inferred mobilized SOC and DOC stocks and fluxes from RTSs from the massof SOC and DOC per meter column in each coastal segment provided in Couture(2010) and Tanski et al. (2016) in relation to the estimated volume ofmaterial displaced by each RTS. The OC values were derived from in situmeasurements collected at 31 locations and were interpolated to each coastalsegment following the same approach as for the determination of ground ice(Couture, 2010). The SOC was measured for different soil unit layers alongthe bluffs and averaged for the upper first meter and lower meter of the soilcolumns (Couture, 2010). It therefore takes into account the heterogeneity ofSOC contents at depth. DOC values account for the differences in DOCconcentrations between wedge ice, massive ice, and non-massive ice (Tanski etal., 2016), based on the ice volumes summarized in Couture and Pollard(2017). The OC values are therefore coarse but consistent for the whole YukonCoast. The dataset is provided in Supplement (Table S1).
3.3.1SOC and DOC stocks
We used Eq. (1) to calculate the stocks of SOC eroded from RTSs:
where RTSSSOC is the stock of SOC eroded from RTSs (expressed inkg),MCTj is the mass of SOC in the upper 1 m (expressed inkg) per coastal segmentj out ofm total,Ai is the total surfacearea of an RTSi out ofn total (expressed in m2),MCBj is the mass of SOC in the lower soil column (expressedin kg) per coastal segmentj, and VSi is the volume of sedimenteroded by per RTS (expressed in m3).MCTj andMCBj take into account differences in dry bulk density percoastal segmentj (Couture, 2010). We used Eq. (2) to calculate the stocksof DOC eroded from RTSs:
where RTSSDOC is the total stock of DOC eroded from RTSs (expressedin kg),Dj is the stock of DOC per coastal segmentj (expressedin kg m−3), and VIi is the volume of ice eroded from a RTS(expressed in m3). Dj is given per coastal segmentj (Tanski etal., 2016).
3.3.2SOC and DOC fluxes
We calculated the flux of material – including ice and sediments – as wellas SOC and DOC fluxes for the RTSs initiated after 1972. To calculate theSOC flux we used Eq. (3):
where RTSFSOC is the annual flux of SOC mobilized from RTSs (expressedin kg yr−1), RTSSSOC is the quantity of SOC eroded from an RTS(expressed in kg) (Eq. 1), and 39 is the number of years during the time period1972–2011. Similarly, we used Eq. (4) to calculate the DOC flux:
where RTSFDOC is the annual flux of DOC eroded from RTSs (expressed inkg yr−1), RTSSDOC is the quantity of DOC eroded from an RTS (expressed inkg) (Eq. 2), and 39 is the number of years during the time period 1972–2011.
4.1Evolution of RTSs between 1952 and 2011
The number of RTSs increased by 73 % between 1952 and 2011. The increasewas more pronounced throughout the time period 1952–1972 (Fig. 5). Between1952 and 2011, active RTSs were more abundant and their number increasedfaster than stable RTSs. While the number of active RTSs progressed steadilythroughout the period, the number of stable RTSs decreased between 1972 and2011: stable RTSs had either been reactivated or been washed away due to coastalretreat. Between 1952 and 2011, the number of RTSs increased by 40 % onlacustrine plains and by 100 % on rolling moraines (Fig. 5). On ice-thrustmoraines, the number of RTSs increased by 69 % between 1952 and 2011 (1.2 RTS yr−1).On both moraine units, the rise was greater between 1952 and 1972.
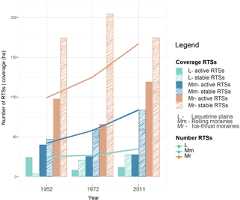
Figure 5Graph showing the evolution in the number and areal coverage of retrogressive thaw slumps (RTSs) between 1952 and 2011 for each geologic units (L, lacustrine plains;Mm, rolling moraines; Mr, ice-thrust moraines). They axis shows both variationsin the number of RTSs and variations in the areal coverage ofRTSs (ha), which differentiate between active and stable RTSs.
The total areal coverage (sum of the total RTS sizes) expanded by 14 %between 1952 and 2011 and was observed in all geologic units (Fig. 5). Thisexpansion was driven by an increase in the areal coverage of stable RTSs(25 %); the areal coverage of active RTSs decreased by 2 % (Fig. 5). Theexpansion in areal coverage was caused by an increase in the number of RTSsrather than by a growth in the size of single RTSs alone: RTSs becamesmaller; their median size decreased by 67 % throughout the period.
Among RTSs present in 2011, 119 initiated after 1972 on previouslyundisturbed surfaces: in 2011, 72 were still active and 47 had stabilized(Table S1). RTSs initiated after 1972 were on average smaller than otherRTSs and occupied 98.6 ha of the whole study area or 22 % of the totalarea affected by RTSs in 2011. Most of the RTSs initiated after 1972(74 %) developed on ice-thrust moraines.
4.2Eroded material and estimated amount of mobilized SOC and DOC
In the following sections, volumes are given for the RTSs that occurredwithin the lidar area. This comprises 56 % of the total number of RTSspresent in the investigated coastal area (n=162) and 41 % of thenumber of RTSs initiated after 1972 (n=49).
4.2.1Eroded material and OC stocks mobilized from RTSs
The total volume of material displaced by the 162 RTSs was16.6×106 m3, 54 % of which was ice (Table S1). It corresponds to avolume of material of0.1×106 m3 km−1 along the YukonCoast. On average each RTS eroded102.2×103 m3 of material.The volume of eroded material was positively correlated to the size of theRTSs (r2=0.5,p<0.05). On average, 52 % of thematerial was displaced due to the retreat of the RTS headwalls and 45 %was transported alongshore due to coastal processes. The remaining 3 % ofmaterial settled in the RTS floors. Overall, 65 % of the material erodedby RTSs originated from ice-thrust moraines, 19 % from lacustrine plainsand 16 % from rolling moraines (Table 1). However, RTSs located onlacustrine plains eroded more material per RTS (135.5×103 m3 RTS−1) than RTSs located onice-thrust moraines (103.9×103 m3 RTS−1) and onrolling moraines (75.1×103 m3 RTS−1).
Table 1Volume of material, including ice and sediments, eroded by RTSsalong the Yukon Coast per geologic unit. The values are normalized to theshoreline length of the geologic units (km).
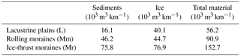
The largest volumes of eroded material came from RTSs occurring at theglaciation limit (Fig. 6). The 24 RTSs located on Herschel Island east(segment 13) eroded 22 % of the total volume of material displaced by the162 RTSs. The RTSs located on Herschel Island west (segment 11) had thehighest volume of material eroded per RTS, on average 4 % of the totalvolume of material displaced by RTSs (Fig. 6). Ice-thrust moraine depositsunderlie both coastal segments 11 and 13.
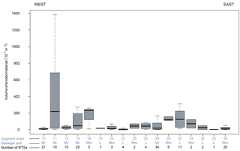
Figure 6Box plot of volumes of eroded material (sediments and ice) per retrogressive thaw slump(RTS) for the coastal segments where RTSs occurred in 2011. Each bar correspondsto a coastal segment, following a geographic order from west on the left toeast on the right. The number of the respective coastal segment is indicatedin the first line on thex axis. The geologic units are indicated below thebars and referred to as L (lacustrine plains), Mm (rolling moraines), and Mr(ice-thrust moraines). The values on the lowest line of thex axis indicatethe total number of RTSs per coastal segment.
Between 1952 and 2011, a total of 162 RTSs displaced7.6×106 m3 of sediments (Table S1), comprising a mass of mineralsediment of6.8×109 kg. On ice-thrust moraines RTSs eroded72 % of the mass of mineral sediments and 19 % on rolling moraines. The total stock of SOC mobilized by RTSs was145.7×106 kg, withthe upper 1 m of soil contributing 49 %. RTSs on ice-thrust morainescontributed to 72 % of the total SOC stock. Out of this, RTSs on HerschelIsland west and east (segments 11 and 13) mobilized 47 % of the total SOCstock. The total stock of DOC mobilized by RTSs was164.5×103 kg. RTSs on ice-thrust moraines mobilized 63 % of the total DOC,which corresponds to103.8×103 kg (Table S1).
4.2.2Eroded material and OC fluxes from RTSs initiated after 1972
The 49 RTSs initiated after 1972 eroded a volume of material of1.1×106 m3, 50 % of which was ice (Table S1). It corresponds to27.2×103 m3 yr−1 (0.6×103 m3 RTS−1 yr−1) between 1972 and 2011. Thisrepresents 6 % of the total volume of material eroded by the 162 RTSs.Most of the material was eroded and transported alongshore due to coastalerosion (67 %). Retreat of the RTS headwalls contributed to 31 % ofthe reworked material from RTSs, and 2 % of material remained in the RTSfloors where it settled.
Table 2Volume of material, including ice and sediments, eroded by RTSsinitiated after 1972 along the Yukon Coast per geologic unit. The values arenormalized to the shoreline length of the geologic units (km).
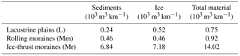
In total, 94 % of the reworked material from RTSs initiated after 1972came from those located on ice-thrust moraines (Table 2), where the largestvolumes of material per RTS initiated after 1972 was23.6×103 m3 RTS−1.The RTSs initiated after 1972 on Herschel Island north(segment 12) reworked the largest volume of material:42.4×103 m3 RTS−1 (Fig. 7).
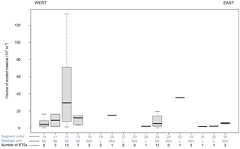
Figure 7Box plot of volumes of eroded material (sediments and ice) per RTSfor the coastal segments where RTSs initiated after 1972 occurred in 2011.Each bar corresponds to a coastal segment, following a geographic order fromwest on the left to east on the right. The number of the respective coastalsegment is indicated in the first line on thex axis. The geologic units areindicated below the bars and referred to as L (lacustrine plains), Mm (rollingmoraines), and Mr (ice-thrust moraines). The values on the lowest line ofthex axis indicate the number of RTSs on the coastal segments.
The 49 RTSs initiated after 1972 eroded a mass of mineral sediments of454.1×106 kg, which represents a flux of11.6×106 kg yr−1. Since 1972, these RTSs mobilized an SOC flux of250.1×103 kg yr−1 (Table 3), representing an average of0.5 kg m−3 yr−1. Most of the SOC fluxes originated from the RTSsinitiated after 1972 on Herschel Island north (segment 12,123.4×103 kg yr−1) and on Kay Point southeast (segment 28,36.8×103 kg yr−1) (Table S1). On ice-thrust moraines, RTSs initiatedafter 1972 mobilized 94 % of the total SOC flux (Table 3). The total DOCflux from RTSs initiated after 1972 was 5.1 kg yr−1, with highvariability between the geologic units: 0.1 kg yr−1 were mobilizedfrom rolling moraines and from lacustrine plains and 4.9 kg yr−1 fromice-thrust moraines (Table S1). The highest DOC fluxes came from ice-thrustmoraines from Herschel Island north (segment 12), where 12 RTSs initiatedafter 1972 mobilized a total flux of 3.1 kg yr−1 of DOC (Table 3).
5.1Increase in slump activity
With a total of 287 RTSs in 2011, the Yukon Coast is one of the Arctic areasmost affected by retrogressive thaw slumping (Ramage et al., 2017). Thenumber of RTSs along the Yukon Coast increased by 73 % between 1952 and2011, with on average two RTSs initiated per year (Fig. 5). The rise was morepronounced between 1952 and 1972 but the number of RTSs continued to increasesteadily between 1972 and 2011. The evolution of RTSs along the Yukon Coastis consistent with the observations made in other parts of the CanadianArctic, where RTS activity has been accelerating since the 1950s (Segal etal., 2016; Lacelle et al., 2010; Lantz and Kokelj, 2008; Lantuit and Pollard,2008). Lantuit and Pollard (2008) showed that the number of RTSs on HerschelIsland increased by 61 % between 1952 and 2000. RTSs develop followingchanges that affect geomorphic settings (Ramage et al., 2017; Kokelj et al.,2017) and are induced by climatic conditions – such as increased airtemperature (Lacelle et al., 2010), precipitation events (Kokelj et al.,2015; Lacelle et al., 2010), and storm events (Lantuit et al., 2012a; Lantuitand Pollard, 2008; Dallimore et al., 1996). Many RTSs that were stable orstabilized between 1952 and 1972 were reactivated between 1972 and 2011. Ourresults confirm the pattern of RTS reactivation previously observed onHerschel Island (Lantuit and Pollard, 2008) and between Kay Point and ShinglePoint (Wolfe et al., 2011), referred to as polycyclicity. Reactivation ofRTSs is associated with the incomplete melting of massive ice bodies duringthe first period of RTS activity (Burn, 2000) and depends on the capacity ofthe slump headwall to remain exposed until ice is exhausted. In coastalsettings, storm events can reactivate RTSs (Lantuit et al., 2012a). Theperiod of RTS activity partly depends on the equilibrium betweenthermo-denudation and coastal erosion rates: the RTS remains active if theRTS headwall erodes at a rate exceeding shoreline retreat (Lantuit et al.,2012a; Are, 1999). This equilibrium is strongly linked to the dynamics ofenvironmental conditions that enhance coastal erosion, such as the occurrenceof storm events and the elongation of the open-water period (Overeem et al.,2011; Are et al., 2008; Solomon, 2005). Climate data recorded at KomakukBeach (segment 2) and Shingle Point (segment 36) show that the average summerair temperature decreased between the periods 1957–1971 (Komakuk,7.4 ∘C; Shingle Point, 10.8 ∘C) and 1971–2000 (Komakuk,4.9 ∘C; Shingle Point, 7.4 ∘C). However, the annual averageprecipitation increased at both stations by 30 and 41 %, respectively,during the same periods (Environment Canada,http://climate.weather.gc.ca/historical_data/search_historic_data_e.html, 2017). Similar patternswere observed for the summer months (July to September). As suggested byKokelj et al. (2015) in other Arctic areas, higher rainfall might intensifyRTS activity. However, a series of environmental factors seems to be jointlyresponsible for the intensification of RTS activity along the Yukon Coast(Ramage et al., 2017).
Along the Yukon Coast, RTSs developed mainly on ice-thrust moraines, wheretheir number increased by 1.1 RTS yr−1 throughout the whole period 1952–2011.Differences in ice content and coastal geomorphology explain the disparitiesin the evolution of RTSs observed among geologic units (Ramage et al., 2017;Lewkowicz, 1987). Our results confirm the results of Kokelj et al. (2017),who showed evidence of a spatial link between RTS occurrence in NorthAmerica and the maximum extent of the Laurentide Ice Sheet. Similar to ourobservations along the Yukon Coast, most of the RTSs in North America arefound along the marginal moraines of the Laurentide Ice Sheet.
Along with the increase in the number of RTSs, the totalareal coverage of RTSs along the Yukon Coast increased by 14 % between 1952 and 2011 (Fig. 5).However, RTSs along the Yukon Coast were on average smaller in 2011 comparedto 1952 and 1972. This differs from RTSs observed in other parts of theCanadian Arctic (Segal et al., 2016; Kokelj et al., 2017). Our resultssupport those reported by Ramage et al. (2017); coastal RTSs are on averagesmaller compared to inland RTSs, and coastal RTSs along the Yukon Coast aresmaller than the ones found in other coastal areas of the Arctic. The largenumber of RTSs initiated after 1972 along the Yukon Coast partly explainsthis: RTSs initiated after 1972 represented 17 % of the total number ofRTSs in 2011; these RTSs were still developing in 2011 and thus had notreached their maximal expansion size.
5.2Eroded material from RTSs and OC fluxes
The expansion of RTSs along the coast causes the displacement of largevolumes of material from the land to the sea. We show that the 56 % ofthe RTSs identified in 2011 for which we could calculate volumes (162 RTSsout of 287 that occur in the coastal area investigated) have reworked atleast16.6×106 m3 of material along the Yukon Coast, whichis102.5×103 m3 RTS−1 of material eroded per RTS(Fig. 6). Among these RTSs, 49 RTSs initiated after 1972 reworked27.2×103 m3 yr−1 of material, which is0.6×103 m3 RTS−1 yr−1 (Fig. 7). These estimates are lowcompared to material removal from other RTSs in the Arctic. Lantuit andPollard (2005) calculated a sediment volume loss of105×103 m−3 between 1970 and 2004 for a single RTS located on HerschelIsland; Kokelj et al. (2015) and Jensen et al. (2014) measured materialdisplacements up to 106 m3 per RTS located in NW Canada andAlaska; the Batagay mega-slump located in Siberia eroded more than24×106 m−3 of ice-rich permafrost in 2014 (Günther et al., 2015).The size of the observed RTSs is one reason behind such differences: most ofthe RTSs examined in the abovementioned studies are classified as mega-slumps(> 0.5 ha). The RTS studied in Lantuit and Pollard (2005) wasthe largest (24 ha) RTS identified along the entire Yukon Coast in 2011.However, most of the RTSs along the Yukon Coast are small, with an averagesize of 0.2 ha (Ramage et al., 2017). This has implications for studies thatattempt to model the impact of RTSs on the eroded material budgets in theArctic.
Couture (2010) estimated the annual flux of mineral sediment eroded byshoreline retreat along the Yukon Coast to7.3×106 kg km−1 yr−1. We showthat along a 190 km portion of the Yukon Coast, 17 % of the RTSsidentified along the coast in 2011 (49 RTSs) contributed to 1 % of theannual flux of material eroded along the Yukon Coast (61×103 kg km−1 yr−1).These RTSs initiated after 1972 incised 1 % (2 km) of the shoreline in2011 and were on average smaller than the average RTSs. Increasing thenumber and areal coverage of coastal RTSs therefore has significant consequenceson the flux of eroded material along the Arctic coasts.
We estimated the annual OC fluxes (SOC and DOC) from these 49 RTSs to be1.3×103 kg km−1 yr−1,including 0.02 kg km−1 yr−1 DOC. The average OC flux fromcoastal retreat along the entire Yukon Coast is157×103 kg km−1 yr−1(Couture, 2010) with an average DOC flux of0.2×103 kg km−1 yr−1 (Tanski etal., 2016). We show that the annual OC flux released by the 49 RTSsinitiated after 1972 was 0.6 % of the annual OC flux from coastal retreat.Most of these fluxes originated from ice-thrust moraines, where the numberof RTSs initiated after 1972 was highest. RTSs develop mainly on ice-thrustmoraines because of the presence of large volumes of massive ground ice(Ramage et al., 2017). As a result, only half of the material eroding fromthe RTS headwall is sediment and most of the OC is released as DOC.
The volumes and stocks of material and OC mobilized by RTSs along the YukonCoast account for 56 % of the RTSs identified on the 2011 imagery. We didnot calculate the volumes for all RTSs present along the Yukon Coast due tothe restricted area covered by the lidar dataset. Therefore, the fluxes ofsediment and OC mobilized by 49 RTSs initiated after 1972 underestimate theannual contribution from RTSs to the nearshore sediment and OC budgets alongthe Yukon Coast. These fluxes account for 41 % of the flux from RTSsinitiated after 1972 and 17 % of the total number of RTSsidentified in 2011 along the Yukon Coast.
5.3Impact of RTSs on the coastal ecosystem
RTSs erode surfaces and scar the landscape, impacting the coastal fringeecosystems. RTSs alter the vegetation composition: after they stabilize,their effects on the vegetation persist over centuries (Cray and Pollard,2015; Lantz et al., 2009). Inland, RTSs modify stream sediment transport byraising the stream turbidity and the concentration of total suspendedsediments (Kokelj et al., 2013). Moreover, RTSs alter coastal retreat (Obuet al., 2016; Lantuit and Pollard, 2008; Leibman et al., 2008) and strongly influence sediment transport along the coast. Segments withintense slumping show the highest volume of eroded and accumulated material(Obu et al., 2016).
Most of the material and OC mobilized through slumping are transported to thenearshore zone (Vonk et al., 2012). However, a fraction of this material andOC remains in the slump floor for several years (Tanski et al., 2017; Obu etal., 2016) where it degrades and is mineralized by microorganisms. Hence, OCis mobilized in RTSs prior to its release to the ocean, which modifies theamount of OC available to the coastal ecosystem (Tanski et al., 2017; Cassidyet al., 2016; Pizano et al., 2014). Tanski et al. (2017) show that SOC andDOC decrease by 77 and 55 %, respectively, before reaching the nearshorezone. Abbott and Jones (2015) describe similar processes for RTSs in uplandareas: after RTSs develop, 51 % of organic-layer SOC and 21 kg m−2of mineral-layer SOC is removed. Following headwall erosion, water transportsmelted ground ice and most sediments to the nearshore zone. Without enoughviscous flow, the remaining part of the sediments accumulates and settles onthe RTS floor, as indicated by higher bulk densities in samples from RTSfloors (Tanski et al., 2017; Lantuit et al., 2012a). The OC in the sedimentsis released to the atmosphere as CO2 (Cassidy et al., 2016), buried inthe RTS floor, or transported to the nearshore zone (Tanski et al., 2017).
RTSs are transient phenomena in coastal settings; coastal retreat eventuallyerodes and transports 45 % of the material reworked by RTSs alongshore.However, as explained above, RTSs affect the OC release process and alterthe OC budget of the nearshore zone.
The number of RTSs along the Yukon Coast increased by 73 % between 1952and 2011, and the total areal coverage of RTSs increased by 14 %. Weobserved disparities between geomorphic units: the largest increase was onice-thrust moraines, where the number of RTSs increased at an annual rate of1.2 RTSs yr−1. Many RTSs are polycyclic and were reactivated between 1972 and 2011.RTSs reworked at least16.6×106 m3 of material within a 190 kmportion of the coastal fringe. The majority of the material came from erosion ofthe headwall (53 %), and 3 % remained in the RTS floors. A large amountof the material from RTSs was eroded and transported alongshore due tocoastal processes (45 %). The OC flux from 17 % of the RTSs identifiedin 2011 was1.3×103 kg km−1 yr−1 and represented 0.6 % of the annual OCfluxes from coastal retreat in the study area. Not all the OC mobilized byRTSs is immediately transported to the nearshore zone; an important part ismobilized in the RTS floors. Therefore, RTSs alter the amount of OC thatenters he nearshore zone by affecting the OC release process. Our resultsshow that the contribution of RTSs to the nearshore OC budget isnon-negligible and should be included when estimating the quantity of OCreleased from the Arctic coasts to the ocean.
The data in this article are available athttps://doi.org/10.1594/PANGAEA.869573(Ramage et al., 2016).
The supplement related to this article is available online at: https://doi.org/10.5194/bg-15-1483-2018-supplement.
JLR and HL designed the study. AMI geocoded the historical photographs usedfor mapping. JLR created the spline interpolation and calculated the erodedvolumes of material from retrogressive thaw slumps. JLR prepared themanuscript with contributions from all coauthors.
The authors declare that they have no conflict of interest.
This article is part of the special issue “Changing Permafrost inthe Arctic and its Global Effects in the 21st Century (PAGE21) (BG/ESSD/GMD/TC inter-journal SI)”.It is not associated with a conference.
This study was supported by the Helmholtz Association through the COPERYoung Investigators Group (VH-NG-801) and by the Alfred Wegener Institute inPotsdam. Justine L. Ramage was financially supported by a PhD stipend by theUniversity of Potsdam. Anna M. Irrgang was financially supported by a PhDstipend from the German Federal Environmental Foundation. We thank Jaroslav Obuand Gustaf Hugelius who provided insight and expertise at an early stage of thestudy.
The article processing charges for this open-access
publicationwere covered by a Research
Centre of the Helmholtz Association.
Edited by: Bo Elberling
Reviewed by: two anonymous referees
Abbott, B. W. and Jones, J. B.: Permafrost collapse alters soil carbonstocks, respiration, CH4, and N2O in upland tundra,Glob. Change Biol., 21, 4570–4587, 2015.
Abbott, B. W., Jones, J. B., Schuur, E. A. G., Chapin III, F. S.,Bowden, W. B., Bret-Harte, M. S., et al.:Biomass offsets little or none of permafrostcarbon release from soils, streams, and wildfire: an expert assessment,Environ. Res. Lett., 11, 034014,https://doi.org/10.1088/1748-9326/11/3/034014, 2016.
Are, F. E.: The role of coastal retreat for sedimentation in the Laptev Sea,in: Land-Ocean Systems in the Siberian Arctic, 288–295,Springer Berlin Heidelberg, 1999.
Are, F. E., Reimnitz, E., Grigoriev, M., Hubberten, H.-W., and Rachold, V.: TheInfluence of Cryogenic Processes on the Erosional Arctic Shoreface, J.Coast. Res., 241, 110–121,https://doi.org/10.2112/05-0573.1, 2008.
Brooker, A., Fraser, R. H., Olthof, I., Kokelj, S. V., and Lacelle, D.: Mappingthe activity and evolution of retrogressive thaw slumps by tasselled captrend analysis of a Landsat satellite image stack, Permafrost Periglac.,25, 243–256, 2014.
Burke, E. J., Hartley, I. P., and Jones, C. D.: Uncertainties in the globaltemperature change caused by carbon release from permafrost thawing, TheCryosphere, 6, 1063–1076,https://doi.org/10.5194/tc-6-1063-2012, 2012.
Burn, C. R.: The thermal regime of a retrogressive thaw slump near Mayo,Yukon Territory, Can. J. Earth Sci., 37, 967–981, 2000.
Burn, C. R. and Zhang, Y.: Permafrost and climate change at Herschel Island(Qikiqtaruq), Yukon Territory, Canada, J. Geophys. Res.-Earth Surf., 114,F02001,https://doi.org/10.1029/2008JF001087, 2009.
Cannone, N., Lewkowicz, A. G., and Guglielmin, M.: Vegetation colonization ofpermafrost-related landslides, Ellesmere Island, Canadian High Arctic, J.Geophys. Res.-Biogeo., 115, G04020,https://doi.org/10.1029/2010JG001384, 2010.
Cassidy, A. E., Christen, A., and Henry, G. H. R.: The effect of a permafrostdisturbance on growing-season carbon-dioxide fluxes in a high Arctic tundraecosystem, Biogeosciences, 13, 2291–2303,https://doi.org/10.5194/bg-13-2291-2016, 2016.
CAVM Team: Circumpolar Arctic Vegetation Map. (1:7,500,000 scale),Conservation of Arctic Flora and Fauna (CAFF), Map No. 1. U.S. Fish andWildlife Service, Anchorage, Alaska, ISBN: 0-9767525-0-6, ISBN-13:978-0-9767525-0-9, 2003.
Couture, N.: Fluxes of Soil Organic Carbon from Eroding Permafrost Coasts,Canadian Beaufort Sea, 155 pp., McGill University, Montreal, Canada, 2010.
Couture, N. J. and Pollard, W. H.: A Model for Quantifying Ground-Ice Volume,Yukon Coast, Western Arctic Canada, Permafrost Periglac., 28, 534–542,https://doi.org/10.1002/ppp.1952, 2017.
Couture, N., Irrgang, A. M., Pollard, W., Lantuit, H., and Fritz, M.:Coastal erosion of permafrost soils along the Yukon Coastal Plain and fluxesof organic carbon to the Canadian Beaufort Sea, J. Geophys. Res.-Biogeo.,123,https://doi.org/10.1002/2017JG004166, 2018.
Cray, H. A. and Pollard, W. H.: Vegetation recovery patterns followingpermafrost disturbance in a Low Arctic setting: case study of HerschelIsland, Yukon, Canada, Arct. Antarc. Alp. Res., 47, 99–113, 2015.
Dallimore, S. R., Wolfe, S. A., and Solomon, S. M.: Influence of ground iceand permafrost on coastal evolution, Richards Island, Beaufort Sea coast,NWT, Can. J. Earth Sci., 33, 664–675, 1996.
Dyke, A. and Prest, V.: Late Wisconsinan and Holocene history of theLaurentide ice sheet, Geogr. Phys. Quatern., 41, 237–263, 1987.
Environment Canada: Canadian Climate Normals 1971–2000 Station Data,available at:http://climate.weather.gc.ca/climate_normals(last access: 18 January 2018), 2017.
Fritz, M., Wetterich, S., Schirrmeister, L., Meyer, H., Lantuit, H.,Preusser, F., and Pollard, W. H.: Eastern Beringia and beyond: lateWisconsinan and Holocene landscape dynamics along the Yukon Coastal Plain,Canada, Palaeogeogr. Palaeocl., 319, 28–45, 2012.
Fritz, M., Vonk, J. E., and Lantuit, H.: Collapsing Arctic coastlines, Nat.Clim. Change, 7, 6–7,https://doi.org/10.1038/nclimate3188, 2017.
Günther, F., Grosse, G., Wetterich, S., Jones, B. M., Kunitsky, V. V.,Kienast, F., and Schirrmeister, L.: The Batagay mega thaw slump, YanaUplands, Yakutia, Russia: permafrost thaw dynamics on decadal time scale,TERRA NOSTRA-Schriften der GeoUnion Alfred-Wegener-Stiftung, 2015.
Hansen, J., Ruedy, R., Sato, M. and Lo, K.: Global surface temperaturechange, Rev. Geophys., 48, RG4004,https://doi.org/10.1029/2010RG000345, 2010.
Harper, J. R.: Morphology of the Canadian Beaufort Sea coast, Mar. Geol.,91, 75–91, 1990.
Huang, J., Zhang, X., Zhang, Q., Lin, Y., Hao, M., Luo, Y., and Nie, S.:Recently amplified arctic warming has contributed to a continual globalwarming trend, Nat. Clim. Change, 7, 875,https://doi.org/10.1038/s41558-017-0009-5, 2017.
Hugelius, G., Strauss, J., Zubrzycki, S., Harden, J. W., Schuur, E. A. G.,Ping, C.-L., Schirrmeister, L., Grosse, G., Michaelson, G. J., Koven, C. D.,O'Donnell, J. A., Elberling, B., Mishra, U., Camill, P., Yu, Z., Palmtag, J.,and Kuhry, P.: Estimated stocks of circumpolar permafrost carbon withquantified uncertainty ranges and identified data gaps, Biogeosciences, 11,6573–6593,https://doi.org/10.5194/bg-11-6573-2014, 2014.
Irrgang, A. M., Lantuit, H., Manson, G. K., Günther, F., and Grosse, G.,Overduin, P. P.: Variability in rates of coastal change along the Yukoncoast, 1951–2015, J. Geophys. Res.-Earth, under review, 2018.
Jensen, A. E., Lohse, K. A., Crosby, B. T., and Mora, C. I.: Variations insoil carbon dioxide efflux across a thaw slump chronosequence in northwesternAlaska, Environ. Res. Lett., 9, 025001,https://doi.org/10.1088/1748-9326/9/2/025001, 2014.
Kessler, L.: Estimating the economic impact of the permafrost carbonfeedback, Clim. Change Econ., 08, 1750008,https://doi.org/10.1088/1748-9326/9/2/025001, 2017.
Kohnert, K., Serafimovich, A., Hartmann, J., and, Sachs T.: Airbornemeasurements of methane fluxes in Alaskan and Canadian tundra with theresearch aircraft Polar 5, Berichte zur Polar-und Meeresforschung=Reports on polar and marine research, 673, available at:http://hdl.handle.net/10013/epic.43357, 2014.
Kokelj, S. V., Zajdlik, B., and Thompson, M. S.: The impacts of thawingpermafrost on the chemistry of lakes across the subarctic boreal-tundratransition, Mackenzie Delta region, Canada, Permafrost Periglac., 20,185–199, 2009a.
Kokelj, S. V., Lantz, T. C., Kanigan, J., Smith, S. L., and Coutts, R.:Origin and polycyclic behaviour of tundra thaw slumps, Mackenzie Deltaregion, Northwest Territories, Canada, Permafrost Periglac., 20,173–184, 2009b.
Kokelj, S. V., Lacelle, D., Lantz, T. C., Tunnicliffe, J., Malone, L., Clark,I. D., and Chin, K. S.: Thawing of massive ground ice in mega slumps drivesincreases in stream sediment and solute flux across a range of watershedscales, J. Geophys. Res.-Earth, 118, 681–692, 2013.
Kokelj, S. V., Tunnicliffe, J., Lacelle, D., Lantz, T. C., Chin, K. S., andFraser, R.: Increased precipitation drives mega slump development anddestabilization of ice-rich permafrost terrain, northwestern Canada, GlobalPlanet. Change, 129, 56–68, 2015.
Kokelj, S. V., Lantz, T. C., Tunnicliffe, J., Segal, R., and Lacelle, D.:Climate-driven thaw of permafrost preserved glacial landscapes, northwesternCanada, Geology, 45, 371–374, 2017.
Koven, C. D., Schuur, E. A. G., Schädel, C., Bohn, T. J., Burke, E. J.,Chen, G., X. Chen, Ciais, P., Grosse, G., Harden, J. W., Hayes, D. J., Hugelius, G., Jafarov, E. E.,Krinner, G., Kuhry, P., Lawrence, D. M., MacDougall, A. H., Marchenko, S. S.,McGuire, A. D., Natali, S. M., Nicolsky, D. J., Olefeldt, D., Peng, S.,Romanovsky, V. E., Schaefer, K. M., Strauss, J., Treat, C. C., and Turetsky, M.: A simplified,data-constrained approach to estimate the permafrost carbon – climatefeedback, Phil. Trans. R. Soc. A, 373, 20140423,https://doi.org/10.1098/rsta.2014.0423, 2015.
Kuhry, P., Dorrepaal, E., Hugelius, G., Schuur, E. A. G., and Tarnocai, C.:Potential remobilization of belowground permafrost carbon under future globalwarming, Permafrost Periglac., 21, 208–214,https://doi.org/10.1002/ppp.684, 2010.
Lacelle, D., Bjornson, J., and Lauriol, B.: Climatic and geomorphic factorsaffecting contemporary (1950–2004) activity of retrogressive thaw slumps onthe Aklavik Plateau, Richardson Mountains, NWT, Canada, Permafrost Periglac.,21, 1–15, 2010.
Lamoureux, S. F. and Lafrenière, M. J.: Fluvial impact of extensiveactive layer detachments, Cape Bounty, Melville Island, Canada, Arct.Antarct. Alp. Res., 41, 59–68, 2009.
Lantuit, H. and Pollard, W. H.: Temporal stereophotogrammetric analysis ofretrogressive thaw slumps on Herschel Island, Yukon Territory, Nat. HazardsEarth Syst. Sci., 5, 413–423,https://doi.org/10.5194/nhess-5-413-2005,2005.
Lantuit, H. and Pollard, W. H.: Fifty years of coastal erosion andretrogressive thaw slump activity on Herschel Island, southern Beaufort Sea,Yukon Territory, Canada, Geomorphology, 95, 84–102, 2008.
Lantuit, H., Pollard, W. H., Couture, N., Fritz, M., Schirrmeister, L.,Meyer, H., and Hubberten, H. W.: Modern and late Holocene retrogressive thawslump activity on the Yukon coastal plain and Herschel Island, YukonTerritory, Canada, Permafrost Periglac., 23, 39–51, 2012a.
Lantuit, H., Overduin, P. P., Couture, N., Wetterich, S., Aré, F.,Atkinson, D., Brown, J., Cherkashov, G., Drozdov, D., Forbes, D. L., andGraves-Gaylord, A.: The Arctic coastal dynamics database: a newclassification scheme and statistics on Arctic permafrost coastlines,Estuar. Coast., 35, 383–400, 2012b.
Lantz, T. C. and Kokelj, S. V.: Increasing rates of retrogressive thaw slumpactivity in the Mackenzie Delta region, NWT, Canada, Geophys. Res. Lett.,35, L06502,https://doi.org/10.1029/2007GL032433, 2008.
Lantz, T. C., Kokelj, S. V., Gergel, S. E., and Henry, G. H.: Relativeimpacts of disturbance and temperature: persistent changes inmicroenvironment and vegetation in retrogressive thaw slumps, Global ChangeBiol., 15, 1664–1675, 2009.
Leibman, M., Gubarkov, A., Khomutov, A., Kizyaakov, A., and Vanshtein, B.:Coastal Processes at the Tabular-Ground-Ice-Bearing Area, Yugorsky Peninsula,Russia, Proceedings of the 9th International Conference on Permafrost,Fairbanks, Alaska, 1037–1042, 2008.
Lewkowicz, A. G.: Headwall retreat of ground-ice slumps, Banks Island,Northwest Territories, Can. J. Earth Sci., 24, 1077–1085, 1987.
MacDougall, A. H., Avis, C. A., and Weaver, A. J.: Significant contributionto climate warming from the permafrost carbon feedback, Nat. Geosci., 5,719–721, 2012.
Mackay, J. R.: Glacier ice thrust features of the Yukon coast, GeographicalBulletin, 13, 5–21, 1959.
Mackelprang, R., Waldrop, M. P., DeAngelis, K. M., David, M. M., Chavarria,K. L., Blazewicz, S. J., and Jansson, J. K.: Metagenomic analysis of apermafrost microbial community reveals a rapid response to thaw, Nature,480, 368–371,https://doi.org/10.1038/nature10576, 2011.
Malone, L., Lacelle, D., Kokelj, S., and Clark, I. D.: Impacts of hillslopethaw slumps on the geochemistry of permafrost catchments (Stony Creekwatershed, NWT, Canada), Chemical Geol., 356, 38–49, 2013.
McGuire, A. D., Koven, C., Lawrence, D. M., Clein, J. S., Xia, J., Beer, C.,et al.: Variability in thesensitivity among model simulations of permafrost and carbon dynam- ics inthe permafrost region between 1960 and 2009, Global Biogeochem. Cy., 30,1015–1037,https://doi.org/10.1002/2016GB005405, 2016.
Obu, J., Lantuit, H., Grosse, G., Günther, F., Sachs, T., Helm, V., andFritz, M.: Coastal erosion and mass wasting along the Canadian Beaufort Seabased on annual airborne LiDAR elevation data, Geomorphology,293, Part B, 331–346,https://doi.org/10.1016/j.geomorph.2016.02.014, 2016.
Olefeldt, D., Goswami, S., Grosse, G., Hayes, D., Hugelius, G., Kuhry, P., and Turetsky, M. R.:Circumpolar distribution and carbon storage of thermokarst landscapes, Nat. Commun., 7,13043, 2016.
Overeem, I., Anderson, R. S., Wobus, C. W., Clow, G. D., Urban, F. E., andMatell, N.: Sea ice loss enhances wave action at the Arctic coast, Geophys.Res. Lett., 38, L17503,https://doi.org/10.1029/2011GL048681, 2011.
Ping, C.-L., Michaelson, G. J., Guo, L., Jorgenson, M. T., Kanevskiy, M.,Shur, Y., Dou, F., and Liang, J.: Soil carbon and material fluxes across theeroding Alaska Beaufort Sea coastline, J. Geophys. Res., 116, G02004,https://doi.org/10.1029/2010JG001588, 2011.
Pizano, C., Barón, A. F., Schuur, E. A., Crummer, K. G., and Mack, M. C.:Effects of thermo-erosional disturbance on surface soil carbon and nitrogendynamics in upland arctic tundra, Environ. Res. Lett., 9, 075006,https://doi.org/10.1088/1748-9326/9/7/075006, 2014.
Ramage, J. L., Konopczak, A. M., Herzschuh, U., Morgenstern, A., Couture, N.,and Lantuit, H.: Coastal retrogressive thaw slumps along the Yukon Coast(Canada), link to shapefile, PANGAEA,https://doi.org/10.1594/PANGAEA.869573, 2016.
Ramage, J. L., Irrgang, A. M., Herzschuh, U., Morgenstern, A., Couture, N.,and Lantuit, H.: Terrain Controls on the Occurrence of Coastal RetrogressiveThaw Slumps along the Yukon Coast, Canada, J. Geophys. Res.-Earth,122, 1619–1634,https://doi.org/10.1002/2017JF004231, 2017.
Rampton, V. N.: Quaternary Geology of the Yukon Coastal Plain, GeologicalSurvey of Canada, Canada, 1982.
Schaefer, K., Lantuit, H., Romanovsky, V. E., Schuur, E. A., and Witt, R.:The impact of the permafrost carbon feedback on global climate, Environ.Res. Lett., 9, 085003,https://doi.org/10.1088/1748-9326/9/8/085003, 2014.
Schneider von Deimling, T., Meinshausen, M., Levermann, A., Huber, V.,Frieler, K., Lawrence, D. M., and Brovkin, V.: Estimating the near-surfacepermafrost-carbon feedback on global warming, Biogeosciences, 9, 649–665,https://doi.org/10.5194/bg-9-649-2012, 2012.
Schuur, E. A., Bockheim, J., Canadell, J. G., Euskirchen, E., Field, C. B.,Goryachkin, S. V., Hagemann, S., Kuhry, P., Lafleur, P. M., Lee, H., andMazhitova, G.: Vulnerability of permafrost carbon to climate change:Implications for the global carbon cycle, AIBS Bulletin, 58, 701–714,2008.
Schuur, E. A. G., McGuire, A. D., Schädel, C., Grosse, G., Harden, J. W.,Hayes, D. J., Hugelius, G., Koven, C. D., Kuhry, P., Lawrence, D. M., andNatali, S. M.: Climate change and the permafrost carbon feedback, Nature,520, 171–179, 2015.
Segal, R. A., Lantz, T. C., and Kokelj, S. V.: Acceleration of thaw slumpactivity in glaciated landscapes of the Western Canadian Arctic, Environ.Res. Lett., 11, 034025,https://doi.org/10.1088/1748-9326/11/3/034025, 2016.
Solomon, S. M.: Spatial and temporal variability of shoreline change in theBeaufort-Mackenzie region, northwest territories, Canada, Geo-Mar. Lett., 25,127–137,https://doi.org/10.1007/s00367-004-0194-x, 2005.
Tanski, G., Couture, N., Lantuit, H., Eulenburg, A., and Fritz, M.: Erodingpermafrost coasts release low amounts of dissolved organic carbon (DOC) fromground ice into the nearshore zone of the Arctic Ocean, Global Biogeochem.Cy., 30, 1054–1068, 2016.
Tanski, G., Lantuit, H., Ruttor, S., Knoblauch, C., Radosavljevic, B.,Strauss, J., Wolter, J., Irrgang, A. M., Ramage, J., and Fritz, M.:Transformation of terrestrial organic matter along thermokarst-affectedpermafrost coasts in the Arctic, Sci. Total Environ., 581, 434–447,2017.
Vonk, J. E., Sánchez-García, L., Van Dongen, B. E., Alling, V.,Kosmach, D., Charkin, A., Semiletov, I. P., Dudarev, O. V., Shakhova, N.,Roos, P., and Eglinton, T. I.: Activation of old carbon by erosion of coastaland subsea permafrost in Arctic Siberia, Nature, 489, 137–140, 2012.
Wolfe, S., Kotler, E., and Dallimore, S.: Surficial characteristics and thedistribution of thaw landforms (1970–1999), Shingle Point to Kay Point,Yukon Territory, Rep., Geological Survey of Canada, 2011.